Research and development efforts in the medical device industry continue to drive innovation toward improving patient care and therapeutic outcomes. Emerging technologies such as biocompatible materials, nanotechnology, and biosensors are at the forefront of revolutionizing healthcare. These advancements demand precise and reliable scientific instrumentation for continued development.
Our suite of cutting-edge tools offers solutions that ensure the development of safe, effective, and innovative medical devices.
Electrospinning and Electrospraying
Electrospinning involves the generation of nano- to microscale fibers typically out of a polymer solution by applying an electric field between a capillary needle and a collector. Similarly, Electrospraying is a technique used to create nano- to microscale particles from a solution or suspension. A wide variety of materials can be processed via these room-temperature techniques, including polymers, metals, ceramics, additives, and live cells. Different materials can be electrospun and electrosprayed simultaneously, enabling the fabrication of complex or layered structures. Fiber and particle diameter, porosity, thickness, mechanical properties, and performance are some of the key aspects both techniques can control.
These techniques play critical roles in research and development for tissue engineering and regenerative medicine, implantable medical devices and biocompatible materials, wound healing and dressings, and drug delivery systems. Examples of these electrospun products are pictured in Figure 1.
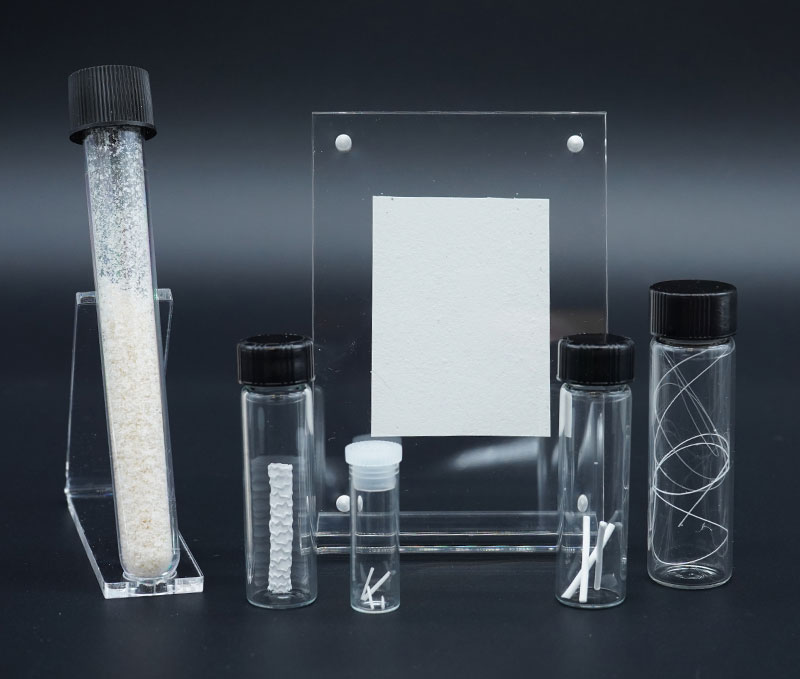
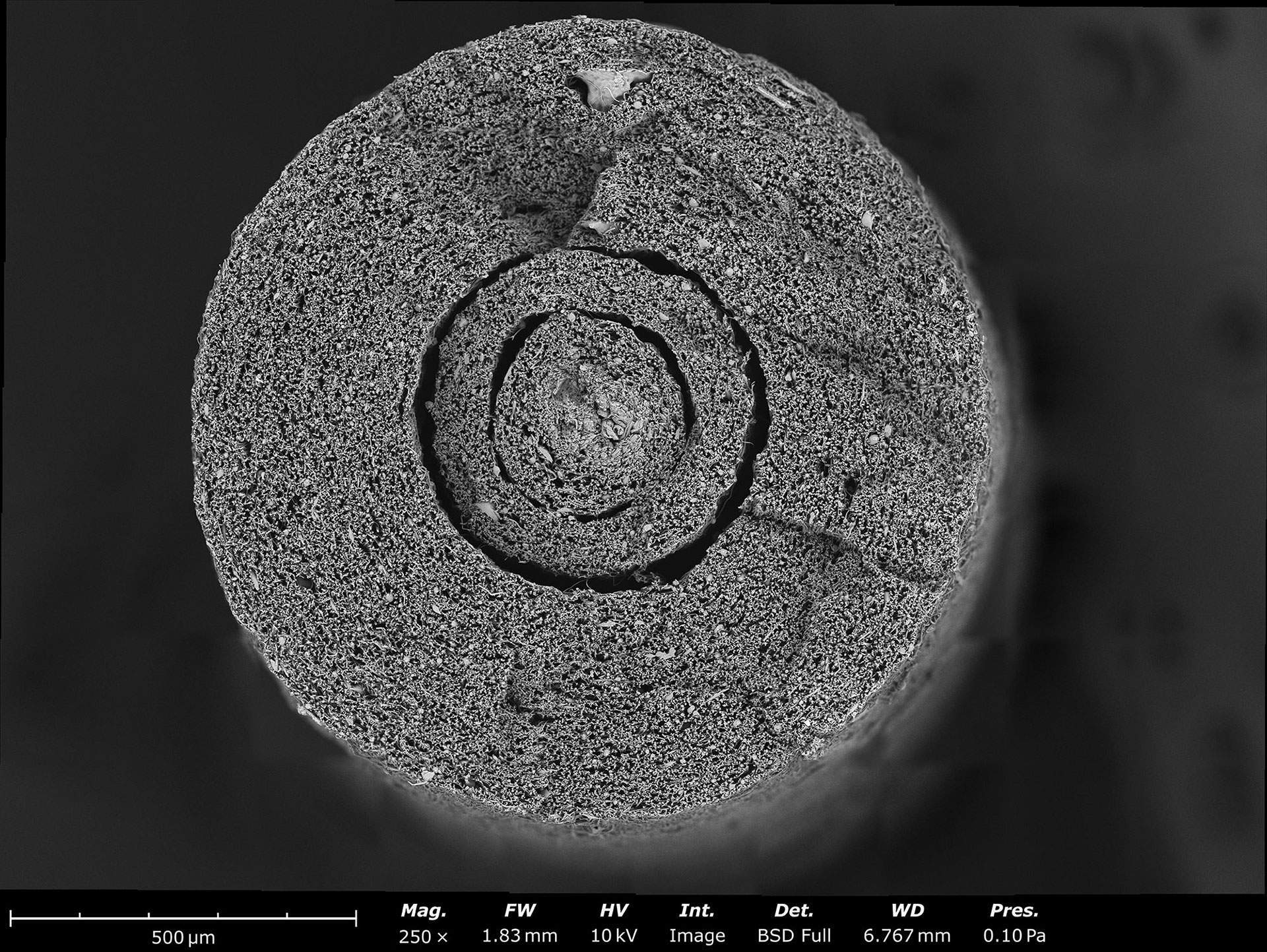
Fabricate Innovative Biomimetic Structures
Electrospinning and electrospraying are used in the field of tissue engineering to fabricate biomimetic scaffolds. These methods enable the fabrication of structures that mimic the extracellular matrix (ECM) of native tissues, promoting cellular adhesion, proliferation, tissue integration, reducing the risk of rejection, encouraging the integration of engineered tissues into the body by supporting cell migration, and accelerating tissue regeneration. Electrospun scaffolds have been applied in various tissue engineering applications such as nerve conduit repair (1, 2), as seen in the Scanning Electron Microscope (SEM) image in Figure 2, bone regeneration (3, 4), and complex 3D structures such as aortic and pharyngeal grafts (5). Electrospun fibers have been used to fabricate biocompatible supports and barriers for various applications such as hernia repair, dural repair, and rotator cuff repair, especially at the tendon-bone interface (6).
Develop Coatings to Enhance Medical Devices
Medical devices can be coated with electrospun fibers or electrosprayed particles to enhance their functionalities. The coatings can be used to modulate the surface and mechanical properties of the medical device. Some examples of this include modifying the hydrophobicity, biofouling, and propensity for cellular adhesion. The fibers and particles can serve as drug-eluting coatings on prefabricated medical devices such as stents (pictured in Figure 3), valves, and occluders (e.g. septal, atrial, vascular) as well as surgical tools such as sutures (7). The properties of the coating can be precisely tuned to optimize the drug elution rates, especially for controlled-release applications (8).
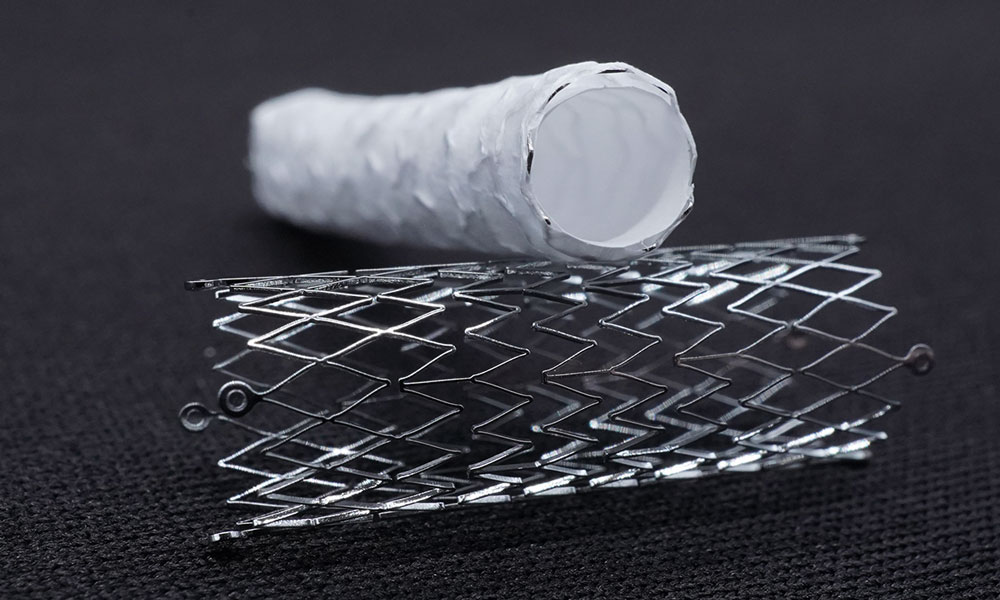
Develop Devices That Accelerate Wound Healing
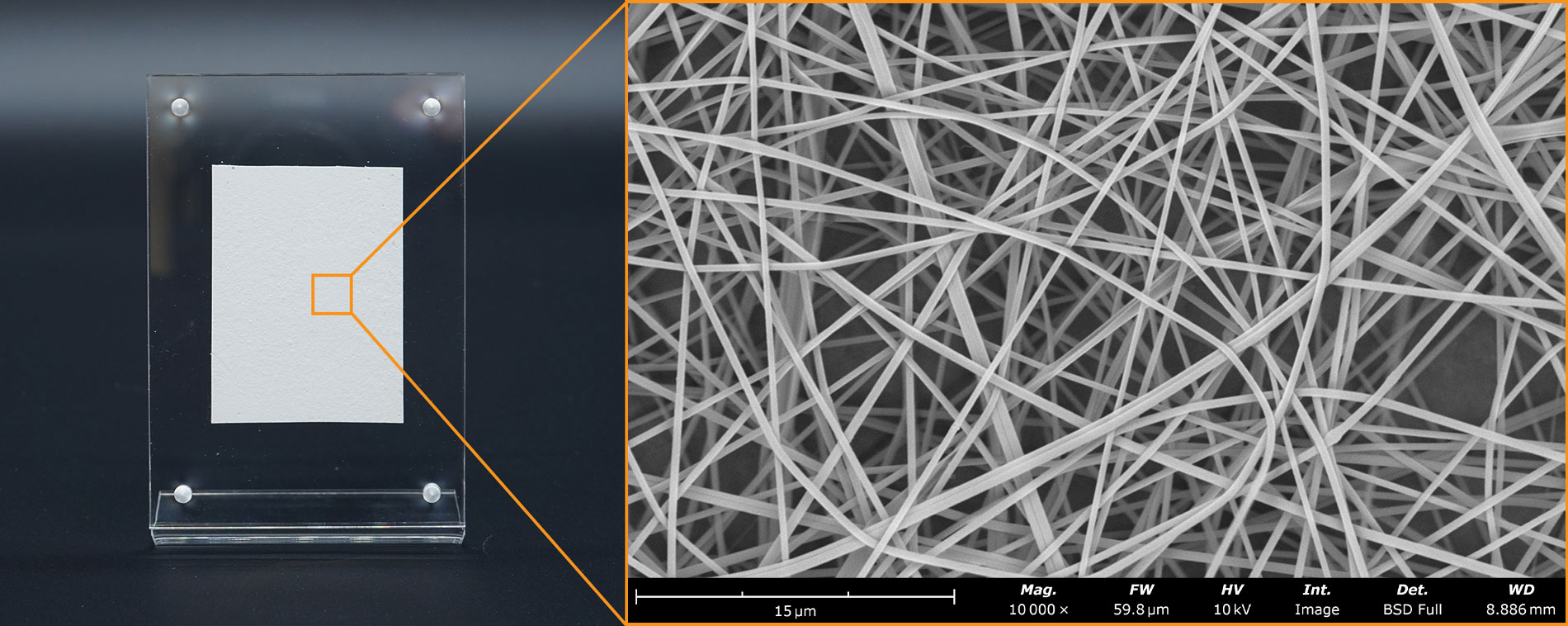
Electrospinning and Electrospraying techniques are increasingly applied in the field of wound healing and dressings, particularly for soft tissue, diabetic ulcers, and burn injuries (9). Electrospun fibers offer unique advantages due to the ability to fabricate structures similar to the ECM of natural tissues. Electrospun dressings provide a scaffold for cell attachment, proliferation, and migration, promoting tissue regeneration and wound closure. The biomimetic ECM structure formed by electrospun fibers facilitates cellular interaction and tissue integration, accelerating the tissue healing process. This microstructure is demonstrated in the SEM image of the commercially-available wound treatment in Figure 4. These wound dressings can also deliver therapeutic agents directly to the wound site such as growth factors, antimicrobial agents, and anti-inflammatory drugs.
Develop Drug Delivery Devices
Electrospinning and electrospraying techniques provide a tailorable platform for the development of drug delivery systems, enabling precise targeting and opening the door to personalized medicine. These fibers and particles can encapsulate therapeutic agents such as drugs, growth factors, or cells, allowing for precise delivery to specific sites within the body. These methods allow the encapsulation of drugs within biocompatible polymers, ensuring targeted drug release, reduced toxicity, and enhanced therapeutic efficacy (10). From antitumoral therapies to intraocular extended-release treatments, electrospun and electrosprayed materials offer tailored solutions to diverse medical needs (11). Additionally, the materials can be engineered to respond to environmental stimuli such as temperature, pH, and electric or magnetic fields.
The Electrospinning and Electrospraying equipment by Fluidnatek consistently delivers batch-to-batch reproducibility of electrospun and electrosprayed nanomaterials. Nanofibrous materials and nanoparticles can be fabricated from a wide variety of FDA-approved synthetic and natural polymers such as PCL, PLGA, PTFE, collagen, gelatin, and hyaluronic acid, opening the door to the fabrication of structures that can reinforce or replace native tissues and other biological structures.
- Control over temperature, relative humidity, and airflow using Environmental Control Units (ECUs)
- Validation for cGMP processes through IQ/OQ/PQ documentation packages
- Established scale-up processes to advance from Research scale to industrial scale production
Characterizing Biomolecular Interactions with QCM-D
Quartz Crystal Microbalance with Dissipation monitoring (QCM-D) is a label-free measurement technique that allows real-time quantification of molecular interactions and processes occurring at surfaces and interfaces. QCM-D typically operates under aqueous conditions, flowing liquid across the surface of a piezoelectric quartz crystal sensor coated with a material of interest to simulate real-world reactions and interactions between molecules. Examples of biological molecules within the detection range for QCM-D are illustrated in Figure 5, (from ~1 Å to 1 um, depending on layer properties). The resulting data provides insights into phenomena such as adsorption, desorption, crosslinking, swelling, and thin film formation, and quantifies viscoelastic layer properties, among other measurable parameters. For these reasons, QCM-D is an invaluable tool for characterizing and quantifying biomolecular interactions, including immune responses to biomaterials and medical implants, antibody-antigen interactions, conformational changes in protein–DNA interactions, nucleation kinetics, and biofouling and biofilm formation.
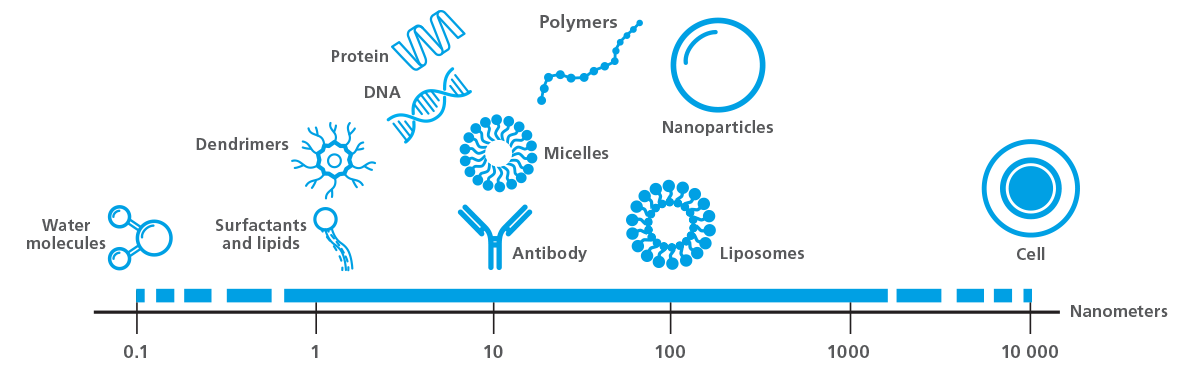
Evaluate Biocompatibility by Simulating Innate Immune Responses
Recent advancements in medical implant and biomaterial technologies have increased their prominence in patient care. This has brought about an advent of material development, with scientists striving to create better materials from which to fabricate implantable devices and biomaterials. However, biocompatibility remains a concern as the human body has several mechanisms in place (i.e. innate immune cascade, foreign body response) to detect and reject materials with incompatible properties.
Therefore, simulating these biological systems and assessing the interactions taking place between the biological molecules and proposed device fabrication materials is paramount to ensuring that they do not trigger adverse reactions in the patient. QCM-D is an ideal tool for these simulations, offering the capability to use relevant surface materials or functional coatings on the QCM-D sensor to investigate biomolecular adsorption, coagulation, cell behavior, and activation markers which are indicative of the triggering of the innate immune system. in a recent study, QCM-D was used to evaluate the protective mechanisms of heparinized cellular and material surfaces against activation of the innate immune response (12).
Design and Evaluate Biocompatible Coatings
Understanding the chemical and physical changes that occur when medical devices encounter biological molecules is crucial for advancing new technologies in healthcare. Materials such as titanium, platinum, silicone, apatite, and some polymers have been studied extensively and are used commonly in medical devices due to their favorable physical and chemical properties for implantation in human patients. However, some of these materials and many others require surface treatments or coatings to enhance their biocompatibility for specific functional purposes. Using these materials as surfaces or coatings on the QCM-D sensor opens the door to in situ, real-time measurement of how they behave in biological systems. The resulting measurements can be interpreted to evaluate thin film formation, layer thickness, rigidity, and subsequent interactions like crosslinking, swelling, calcification, and drug release. QCM-D data can also provide insights into protein adsorption, cell attachment, immune responses, and the success of surface modifications. An experiment of this nature that aimed to study specific functionalization of biomaterials via surface modification is illustrated in Figure 6, in which multilayer polyelectrolyte films were creating using layer-by-layer deposition and subsequently tested for specific and nonspecific protein adsorption (13).
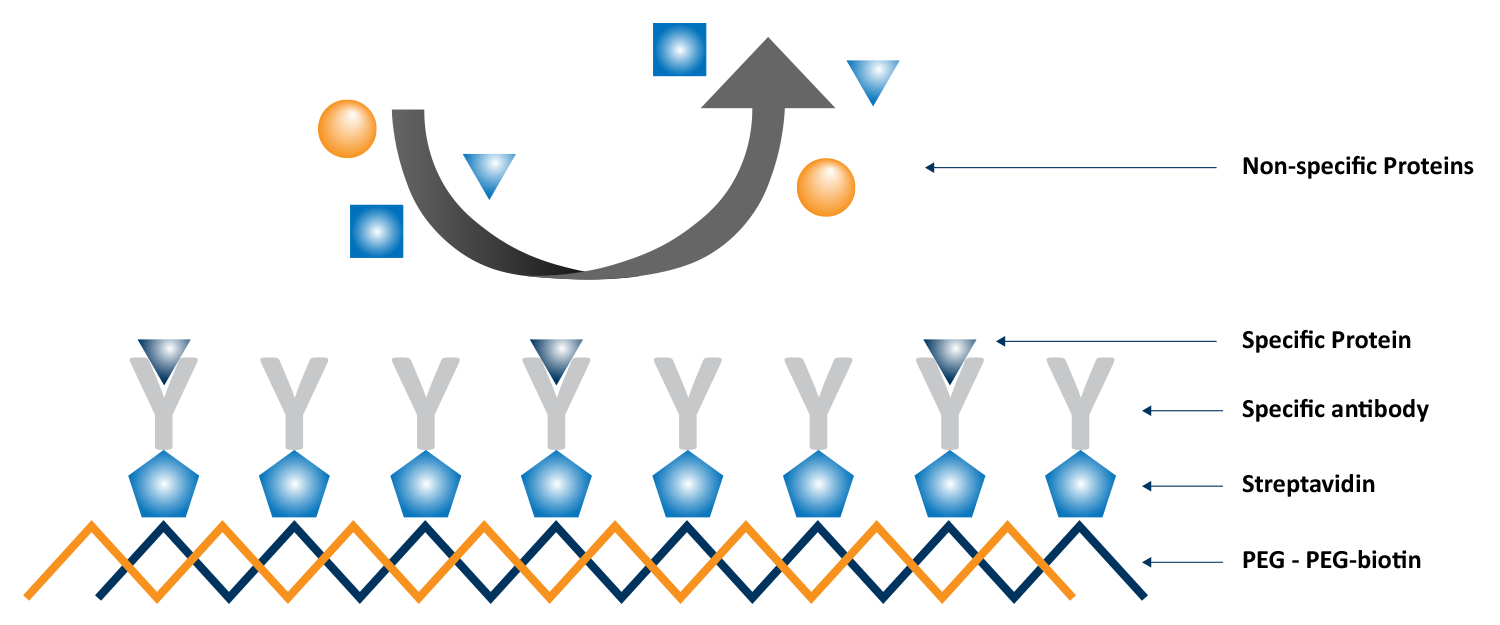
- Characterization and evaluation of biocompatibility:
- Characterization of cell adhesion and spreading in real time
- Characterization of biomineralization:
Develop Anti-fouling Coatings
Biofilms are microbial communities that adhere to surfaces. Fouling induced by extracellular polymeric substances secreted by the microorganisms that comprise the biofilm significantly impact membranes and other materials used in medical devices. While cleaning methods for fouled membranes and materials have been well-studied, strategies for preventing fouling provide a more desirable and economical solution. QCM-D is an excellent tool for understanding and characterizing conditions that minimize and prevent fouling and biofilm formation, which are crucial for ensuring optimal long-term performance of these medical devices and products. The data in Figure 7 demonstrate how QCM-D was used to detect early biofilm formation on the surface of stainless steel, which is a common material used in implantable medical devices. Using QCM-D, it is possible to collect data that enhances understanding of the relationship between surface properties and the material’s propensity to fouling (14). QCM-D experiments also facilitate the characterization of scale buildup, fouling processes, and detection of biofilm formation by measuring bacterial attachment and growth in real time. In addition, QCM-D technology aids in evaluating antiscalant and antifouling strategies, providing critical information for the design of effective antifouling membrane coatings.

Characterization of biofouling, biofilm formation, and antifouling coatings
Biosensor to Detect Nanoscale Mass Changes
The biological detection systems within biosensors are layered and configured with diverse building blocks of receptors such as antibodies, proteins, DNA, cells, lipid-based structures, carbohydrates, and nanoparticles, among others. In addition to the bioreceptor, biosensors require a transducer to convert the biorecognition event to a measurable signal. When using QCM-D as a biosensor, the working principle of the transducer is acoustic. Operating on the piezoelectric principle, QCM-D detects nanoscale mass and viscoelastic changes in situ, providing detailed information about the processes being measured at the surface of the QCM sensor. This approach has been successfully implemented for the detection of analytes and quantification of a wide variety of biological processes, including heavy metal ion detection, DNA hybridization, cell attachment, proliferation, and growth, and cell responses to external stimuli. An example of a QCM-D-based assay is illustrated in Figure 8, which was used to study antibody-antigen interactions using biotin-functionalized sensors as the base of the assay. The range of detection for QCM-D is expansive, with ongoing exploration of sensor interfacial strategies to enhance sensitivity and selectivity for specific analytes.
QSense QCM-D as an acoustic biosensor
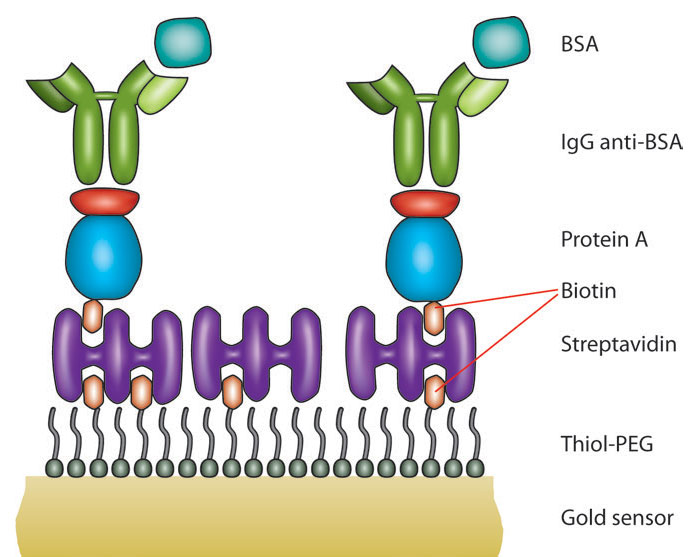
The QSense Omni is the latest advancement in QCM technology from the pioneers of the QCM-D technique. With the highest sensitivity of any QCM available on the market, the Omni enables the quantification and monitoring of faster processes involving smaller molecules, which is ideal for investigating biological processes. Over 100 sensor surface materials and coatings are available, supporting the simulation of real biological environments and processes to characterize protein adsorption rates, thin film formation, layer rigidity, calcification, cell attachment, and much more.
- Resolve smaller changes at the sensor surface down to 0.24 ng/cm2
- Automated features to minimize user-dependent data variation
- Intuitive software with easy workflows
Optical Tensiometry:
Optical Tensiometry is a technique used to measure the surface and interfacial tension of liquids and solid surfaces. The technique relies on the shape of a droplet of liquid on the surface which can be fit with an appropriate algorithm to deduce information about the intermolecular forces acting at the interface between the droplet and the surface including surface tension and wetting properties.
Optical tensiometry is an excellent tool for characterizing wettability and offers a flexible platform for accommodating samples over a wide range of shapes and sizes, including soft polymeric biomaterials and unique 3D shapes. Various contact angle methods including static and dynamic contact angle, as well as surface free energy (SFE), can be used to elucidate the surface properties of a material of interest and predict how they will interact with the biological environment. In some cases, additional characterization techniques are necessary to accurately represent the physical and chemical properties of a surface material. Roughness-corrected contact angle measurements use simultaneous optical profilometry during contact angle measurements to correct the contact angle for irregular surface topography, which is of particular interest for characterizing the wettability of medical implants that are surface treated or roughened to improve cell attachment and general biocompatibility.
Assessing the Biocompatibility of Medical Devices
Biocompatibility is an ever-increasing issue as new materials are developed for therapeutic applications. The biocompatibility of the surface of the medical device that interacts with the various bodily fluids is critical to the success of these devices. The biocompatibility of the device is influenced by its surface characteristics, particularly the surface roughness, surface chemistry, and wettability. From bacterial adhesion and biofouling to cell attachment and osseointegration, these surface characteristics determine whether the device will be integrated or rejected. Using tensiometry methods, it is possible to fully characterize all relevant surface characteristics to assess whether a material is a viable candidate for use as a medical device.
In the example in Figure 9, an artificial skin sample was tested using roughness-corrected contact angle to determine the surface roughness of the material while simultaneously measuring the contact angle to assess its wettability and topography. Through subsequent testing, the roughness-corrected surface free energy of the material can be determined, which provides insight into the effects of various surface properties (chemistry, topography, etc.) on whether proteins, cells, and other molecules in the biological environment will adhere to the surface.
- Wettability measurements in biomedical applications: AT WP Wettability measurements in biomedical applications.pdf (biolinscientific.com)
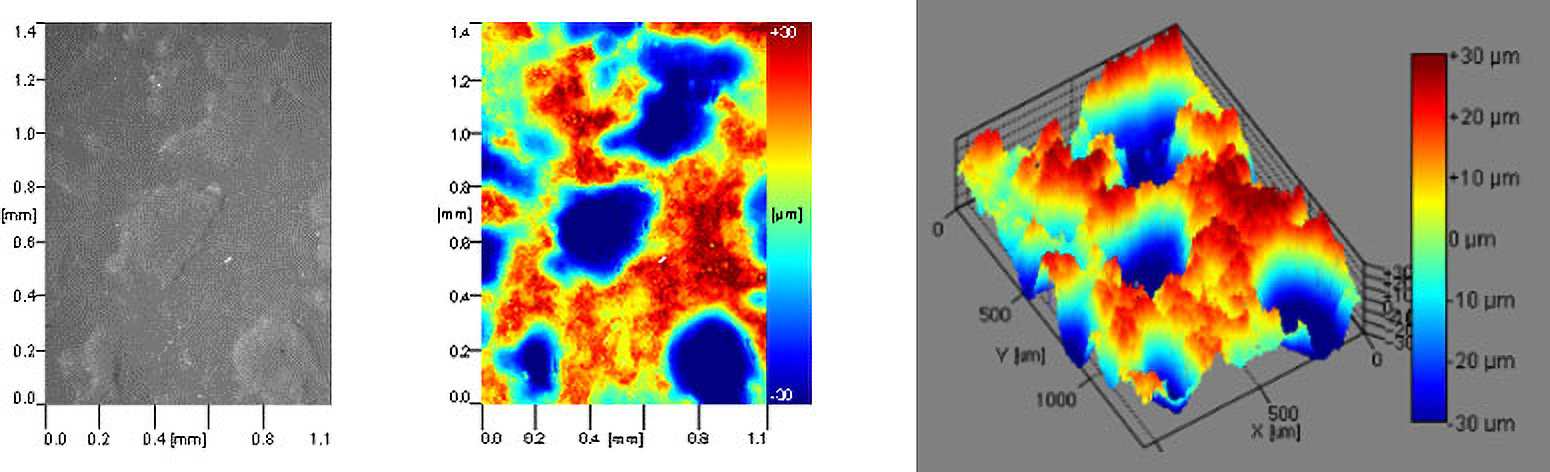
Characterizing Wettability of Small Medical Devices
Manufacturers are working to miniaturize medical devices, making them less invasive to improve patient recovery timelines and reduce unwanted immune responses. However, as the surface areas on these devices shrink, it becomes more challenging to characterize them properly as a typical droplet used to measure contact angle and its related wettability characteristics are too large to sit on the surface of the material of interest. In cases like these, it is imperative to find a better characterization solution to ensure biocompatibility studies and quality control can proceed according to the demanding standards of the medical device industry. Biolin Scientific has commercialized an innovative technology that enables the dispensing of picoliter-volume droplets to measure contact angle on small surfaces, including medical tubing, single biomaterial fibers, down to individual frets on stent implants. In Figure 10, there are three examples of contact angle measurements on very small devices captured by the Attension Theta Pico optical tensiometer: a biomaterial fiber with a diameter of approximately 110 µm, an 8mm internal diameter (ID) stent with ~80 µm wire, and a 6mm ID stent that was coated with polycaprolactone (PCL), an FDA approved polymer, via electrospinning.
Figure 10: Contact angle measurements using picoliter-volume droplets on a biomaterial fiber (a), an uncoated stent (b), and an electrospun polycaprolactone (PCL) coated stent (c).
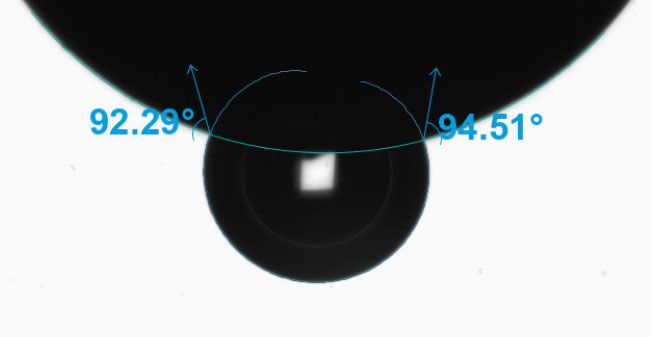
The Attension Theta Flow automates several aspects of contact angle experiments including camera focus, stage position, drop placement, image capture, and drop fitting, ensuring accurate data across multiple experiments and users. Common wettability characterization methods are available as pre-programmed recipes, accelerating the process from start to finish. The roughness-corrected contact angle is supported through an optional optical profilometry module, ensuring contact angle measurements are accurate regardless of the material’s surface topography or surface treatments. With one Attension optical tensiometer, a wide variety of measurement methods are possible, including standard contact angle (also referred to as sessile drop), surface free energy, dynamic contact angle, inverted bubble contact angle (pictured in Figure 11), dilatational interfacial rheology, roughness-corrected contact angle, and more.
- All-inclusive OneAttension software provides immediate and unlimited access to all contact angle measurement methods
- Highest resolution camera with autofocus, highest frame rate, and largest field-of-view
- Ultimate accuracy/traceability of measurement data thanks to integrated sensors for temperature, camera tilt, ambient humidity, and levelness of the instrument
- Reduce user bias with pre-programmable experiment recipes, automated droplet placement, and real-time automatic data analysis
References
- Development of a Piezoelectric PVDF-TrFE Fibrous Scaffold to Guide Cell Adhesion, Proliferation, and Alignment
- Development of ibuprofen-loaded electrospun materials suitable for surgical implantation in peripheral nerve injury
- Incorporation of Superparamagnetic Iron Oxide Nanoparticles into Collagen Formulation for 3D Electrospun Scaffolds
- Bone morphogenetic protein (BMP)-modified graphene oxide-reinforced polycaprolactone — gelatin nanofiber scaffolds for application in bone tissue engineering
- Patient-Specific 3-Dimensional Model of Smooth Muscle Cell and Extracellular Matrix Dysfunction for the Study of Aortic Aneurysms
- Fabrication of hybrid scaffolds obtained from combinations of PCL with gelatin or collagen via electrospinning for skeletal muscle tissue engineering
- Development of Ciprofloxacin-Loaded Electrospun Yarns of Application Interest as Antimicrobial Surgical Suture Materials
- Coaxial electrospinning of polycaprolactone – A design of experiments approach
- Design of a composite wound dressing: Combining an electrospun fleece with a free-standing multilayer film
- Release of amoxicillin and doxycycline from PLA nanofibers optimized using factorial experimental design
- Mucoadhesive brinzolamide-loaded nanofibers for alternative glaucoma treatment
- Regulation of the innate immune system by fragmented heparin-conjugated lipids on lipid bilayered membranes in vitro
- Control of Specific/Nonspecific Protein Adsorption: Functionalization of Polyelectrolyte Multilayer Films as a Potential Coating for Biosensors
- Study of the Human Albumin Role in the Formation of a Bacterial Biofilm on Urinary Devices Using QCM-D